Nucleic acids – Structure and physico-chemical properties
Sakshi Education

From the time of discovery of nucleic acids by Fredrick Miescher in 1870, they were long regarded as something of a curiosity until the structures of the monomer units, the nucleotides, was established in 1909 and that of RNA was proposed by Levene and Tipson in 1935. Nucleic acids are basically of two types- DNA and RNA, which are polymers of nucleotide chains. Each nucleotide comprises of three parts, firstly - a sugar moiety which is a pentose (five-membered-ring) joined to a second part-phosphate groups. The anomeric carbon of each sugar is bonded to the nitrogen atom of a third part - heterocyclic compound in a ß- glycosidic linkage. The linkage is named so, as the substituents at C-1 and C-4 are on the same side of the furanose ring.
As the heterocyclic compounds which formed a part of the nucleic acids contained amine groups and in general amines act as bases, these heterocyclic compounds were also arbitrarily referred to as bases. Moreover, since the hetero atom in the ring is Nitrogen- these compounds were named as Nitrogen Bases. Nitrogen bases are planar, aromatic, heterocyclic molecules which for the most part are derivatives of purine or pyrimidine. There are only four bases in DNA—two are substituted purines (adenine and guanine), and two are substituted pyrimidines (cytosine and thymine). RNA also contains only four bases. Three (adenine, guanine, and cytosine) are the same as those in DNA, but the fourth base in RNA is uracil instead of thymine. Thymine and uracil differ only by a methyl group—thymine is 5-methyluracil.
Why do Nucleic acids contain only Ribose or Deoxy-Ribose sugars ?
In RNA the five-membered-ring sugar is D-ribose. In DNA it is 2’-deoxy-D-ribose (D-ribose without an OH group in the 2-position). The choice of five membered ring is by default as these rings are the most stable amongst aromatic compounds except in the case of benzene, a six membered ring which is stabilized by resonance. Amongst the pentoses, Ribose is selected during evolution, as the exclusive sugar component of nucleic acids. The selection is explained by using molecular models and by eliminating most of the other common sugars by looking at their chemical structure and envisioning how they would fit in a nucleic acid model. Comparisons of sugar pucker conformations and configurations of pentoses indicate that ribose was not randomly selected but the only choice, since ß-D-ribose fits best into the structure of physiological forms of nucleic acids. In other nucleotides containing arabinose, xylose, or lyxose, the C2'-OH and/or the C3'-OH are above the furanose ring, causing steric interference with the bulky base and the C5'-OH group.
Why Nucleic acids are negatively charged?
Phosphoric acid links the sugars in both RNA and DNA. The acid has three dissociable OH groups with pKa values of 1.9, 6.7, and 12.4. Each of the –OH groups of the phosphoric acid is equally capable of reacting with an alcohol (acid + alcohol -> ester) to yield a phosphomonoester, a phosphodiester, or a phosphotriester. In nucleic acids the phosphate group forms only a phosphodiester. Although, the phosphoric acid is capable of forming three ester bonds, it is forming only two bonds, leaving the third –OH group free, which upon dissociation imparts negative charge. In case of RNA, the additional 2’-OH group also adds to the negative charge. Therefore RNA has more charge and is therefore less stable and exhibits greater mobility upon electrophoresis than DNA.
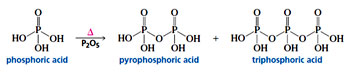
Heating of phosphoric acid molecules results in formation of pyrophosphoric acid, which is a phosphoanhydride. formed by loss of a water molecule. The name pyrophosphate is derived from the the Greek word - pyr, which means “fire.” Thus, pyrophosphoric acid is prepared by “fire”—that is, by heating. In the above said manner, Triphosphoric acid and higher polyphosphoric acids can also be formed, due to the ability of phosphoric acid to form an anhydride.
Nucleotides can exist as monophosphates, diphosphates, and triphosphates and are named by adding monophosphate or diphosphate or triphosphate to the name of the nucleoside. These phosphoanhydride bonds are High energy bonds which means that when these bonds are broken , they release lot of energy, which can be simultaneously used for driving other chemical reactions in the cell.
The purines and pyrimidines are bonded to the anomeric carbon of the furanose ring—purines at N-9 and pyrimidines at N-1—in a -glycosidic linkage. A compound containing a base bonded to D-ribose or to 2’-deoxy-D-ribose is called a Nucleoside. In a nucleoside the ring positions of the sugar are indicated by primed numbers to distinguish them from the ring positions of the base. This is why the sugar component of DNA is referred to as 2’-deoxy-D-ribose. For example, adenine is the base, whereas adenosine is the nucleoside. Similarly, cytosine is the base, whereas cytidine is the nucleoside, and so forth. Uracil is found only in RNA and is therefore is attached to D-ribose but not to 2-deoxy-D-ribose. Similarly, because thymine is found only in DNA, it is attached to 2-deoxy-D-ribose but not to D-ribose.
A nucleotide is a nucleoside with either the 5’ or the 3’ -OH group bonded in an ester linkage to phosphoric acid. The nucleotides of RNA—where the sugar is D-ribose—are more precisely called ribonucleotides, whereas the nucleotides of DNA—where the sugar is 2-deoxy-D-ribose—are called deoxyribonucleotides.
Biological functions of Nucleotides:
Nucleotides apart from acting as integral units of DNA and RNA, tperform various cellular activities such as donors of chemical energy, secondary messengers in cell signalling, cofactors for enzymes, as vasidialtors, carriers of subunits required in cell wall synthesis and lipid biosynthesis.
ATP- Currency of the cell:
All living systems need energy to ensure their survival and reproduction and they derive energy by converting nutrients into a chemically useful form of energy. The most important form of chemical energy is adenosine -triphosphate (ATP). The importance of ATP to biological reactions is shown by its turnover rate in humans—each day, a person uses an amount of ATP equivalent to his or her body weight. ATP is known as the universal carrier of chemical energy as it is commonly used in all living systems from prokaryotes to eukaryotes. ATP has the ability to convert thermodynamically unfavorable reactions to favorable ones as “the energy of hydrolysis of ATP converts endergonic reactions into exergonic reactions.”
The secret behind the stability of ATP:
As it can be noticed from the structure of ATP, that it is highly negatively charged as any nucleotide triphosphate has four units of negative charge and must be highly reactive and thereby making it less stable. Considering the above fact, although ATP reacts readily in enzyme-catalyzed reactions, but surprisingly, its reaction rate is quite slow in the absence of an enzyme. When we compare with carboxylic acid anhydrides which hydrolyze in a matter of minutes, ATP takes several weeks to hydrolyze. The low rate of ATP hydrolysis is a favourable consequence for the cell as it can be retained in the cell for a longer period of time and be available when needed for an enzyme-catalyzed reaction. It is very interesting to know that the reason behind slow reactivity of ATP in the absence of enzymes is its negative charge which repels the approach of nucleophiles. When ATP is bound at an active site of an enzyme, it complexes with magnesium which decreases the overall negative charge on ATP. This is why ATP-requiring enzymes also require metal ions; The other two negative charges can be stabilized by positively charged groups such as arginine or lysine residues at the active site. In this form, ATP is readily approached by nucleophiles, so ATP reacts rapidly in an enzyme-catalyzed reaction, but only very slowly in the absence of the enzyme.
Other Important Nucleotides and teir functions:
dTMP is formed when dUMP is methylated, with coenzyme -methylenetetrahydrofolate supplying the methyl group. Because the incorporation of the methyl group into uracil oxidizes tetrahydrofolate to dihydrofolate, dihydrofolate must be reduced back to tetrahydrofolate to prepare the cofactor for another catalytic cycle.
The reducing agent is NADPH and every NADPH produced in the cell can generate three ATPs. Based on the this fact, the usage of NADPH to reduce dihydrofolate comes at the expense of ATP. Although the process of synthesis of thymine is energetically expensive still the cell prefers to recruit Thymine in DNA rather than Uracil.
The presence of uracil in DNA is mutagenic and presence of thymine prevents potentially lethal mutations. The chemical phenomenon behind this is chemical instability of Cytosine which can tautomerize to form an imine, which subsequently gets hydrolyzed to uracil. The overall reaction is called a deamination since it removes an amino group.
If a cytosine in DNA is deaminated to a uracil, uracil will specify incorporation of an adenine into the daughter strand during replication instead of the guanine that would have been specified by cytosine. This would have adverse effects as it leads to changes in the nucleotide sequence and can cause GC basepairs to AT base pair mutations. Inorder to avoid such complications presence of a U in DNA is recognized as a “mistake” by celllular enzymes before an incorrect base can be inserted into the daughter strand. Uracil-N-glycolsylase and dUTPase are the two enzymes which ensure the elimination of U from DNA. dUTPase cleaves UTP molecules present in the nucleus and prevents them for getting into the active site of DNA polymersase. In case of any U being incorporated into the DNA, Uracil-N-glycolsylase cleaves the glycosydic bond and removes the nucleotide frorn DNA. This generates a apyrimidinic site which is recognized as a lesion and is further repaired by repair enzymes.
If U’s were normally found in DNA, the enzymes could not distinguish between a normal U and a U formed by deamination of cytosine. Having T’s in place of U’s in DNA allows the U’s that are found in DNA to be recognized as mistakes. Unlike DNA, which replicates itself, any mistake in RNA does not survive for long because RNA is constantly being degraded and then resynthesized from the DNA template. Therefore, it is not worth spending the extra energy to incorporate T’s into RNA.
Properties of nucleic acids:
Effect of temperature
Duplex nucleic acid structures get disrupted or denatured at high temperature into single stranded molecules and the process is termed as ‘melting’, the process of melting of double helices can be monitored easily because base stacking and base-pairing interactions significantly reduce UV absorption. As the temperature of a solution containing a polynucleotide duplex is raised, the absorption of UV light will greatly increase around the temperature at which the two strands separate into single-stranded polynucleotides. This is due to the fact that single stranded molecules exhibit higher UV absorption than the double stranded molecules at a wave length of 260 nm. The transition in UV absorption typically occurs over a 48?C to 88?C range. When a plot is drawn with the Absorbance on X-axis and Temperature on Y-axis, it is termed as Melting curve. The midpoint of the transition or melting curve at which half of the polynucleotide species is duplex and half is single-stranded is identified as the Melting temperature (Tm). In general, DNA duplexes are relatively less stable to thermal denaturation than RNA duplexes or RNA-DNA duplexes.
Hyperchromic shift: Increase in the absorption at 260nm upon denaturation of double stranded DNA resulting in formation of single stranded DNA is termed as Hyperchromic shift.
Hypochromic shift: Decrease in the absorption at 260nm upon renaturation of single stranded DNA molecules to yield double stranded DNA is termed as Hypochromic shift. Renaturation bt slow cooling is called Annealing.
The Tm for a given duplex is otherwise affected by a number of factors. The major determinants include sequence length, G-C content and salt concentration. Longer duplexes or those having a higher proportion of G-C pairs are more stable and therefore will have a higher Tm. Monovalent cations, such as sodium (Na+) and potassium (K+), stabilize double helices by partially neutralizing the negative charge along the phosphodiester backbone. Consequently, higher salt concentration will also increase the Tm of a given polynucleotide duplex. In addition the presence of mismatched bases in a duplex will generally have a destabilizing effect and decrease Tm.
Properties that get altered upon Denaturation:
Effect of pH on nucleic acids:
Nucleic acids are stable between a pH range of 5-9. Beyond this range, they undergo denaturation spontaneously due to the tautomeric shift of Nitrogen bases altering the H- bonding between the nucleotides of the opposite strands.
Effect of Acid on nucleic acids:
Both DNA and RNA undergo acid hydrolysis. The glycosidic bonds of purine nucleosides are susceptible to acid hydrolysis by protonation at N7 of guanosine or adenosine. Under mild acidic conditions DNA becomes Apurinic DNA. Depurination of DNA is more favourable than that of RNA, although the formation of an abasic site in the polynucleotide results in either case. A complete loss of sequence information represented by an abasic site in DNA can likewise cause a permanent mutation.
Effect of Alkali on nucleic acids:
RNA undergoes alkali hydrolysis due to the presence of 2’-OH group but DNA doesn’t undergo alkali hydrolysis.
Although both RNA and DNA can be degraded through cleavage of the phosphodiester backbone, their chemical stability is drastically different. RNA is distinctively subjected to a transesterification reaction in which the C2’ oxygen serves as a nucleophile for attack on the adjacent phosphorus. This particular reaction proceeds through a pentacoordinate phosphate intermediate, in which the bond formed between the C2’ oxygen and the phosphorus centre which is in line with the bond to be broken between the phosphorus centre and the C5’ oxygen. As a aresult, the reaction products terminate with a C5’ hydroxyl and C2’, C3’-cyclic phosphate. This cyclizing mechanism of RNA transesterification occurs easily because the nucleophile is inherently positioned in proximity to the phosphorus centre in the phosphodiester backbone. Moreover, the reaction is promoted either by deprotonation of the C2’ hydroxyl or protonation of the C5’ oxygen. Therefore, RNAs are easily degraded in alkaline or acidic solutions. A similar mechanism applicable to both RNA and DNA polymers, operates for cleavage of phosphodiester , in which a second molecule serves as the attacking nucleophile. The reaction is similarly promoted by deprotonation of the attacking group and protonation of the leaving group. However, the products terminate with a C5’ phosphate and C3’ hydroxyl. Water can serve as a nucleophile in the spontaneous hydrolytic degradation of RNA or DNA polymers, although the reaction is approximately 100 000 times less likely to occur than RNA transesterification. Based on the above principle and the fact that each sugar lacks a C2’ hydroxyl group, the phosphate and sugar backbone of DNA exhibits a relatively huge chemical stability. In fact, DNA is the most stable of the biological polymers enabling it to maintain the integrity of genetic information- a prerequisite to act as genetic material.
Effect of bisulfite and nitrous acid:
Bisulfite and nitrous acid promote deamination or removal of an amino group from cytosine, resulting in conversion of cytosine to uracil. In DNA, cytosine deamination will ultimately lead to conversion of a G-C base pair to an A-T base pair if left uncorrected.
Effect of Ionizing radiations and Oxidizing agents:
Strong oxidizing agents such as hydroxyl radicals generated by ionizing radiation or incomplete metabolic reduction of oxygen producing superoxide ions, hydrogen peroxide or hydroxyl radicals react extremely with DNA. Hydroxyl radicals can nonspecifically damage DNA by breaking the phosphodiester backbone. Strand scission occurs by a number of mechanisms, each of which produces polynucleotide fragments with different 5’and 3’ terminal products. In addition, hydroxyl radicals can directly attack and alter the chemical composition of the bases in nucleic acids generating compounds like 8-hydroxyguanine and thymine glycol. These modifications result in formation of lesions in the sequence of DNA making them susceptible to mutations, which may ultimately lead to cancer. Therefore, ionizing radiation and other compounds that cause DNA damage are typically carcinogenic or cancer causing.
Forces stabilizing nucleic acid structure:
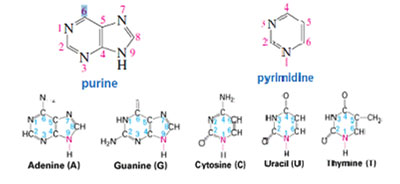
Why do Nucleic acids contain only Ribose or Deoxy-Ribose sugars ?
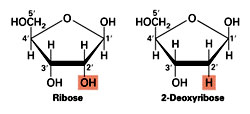
Why Nucleic acids are negatively charged?

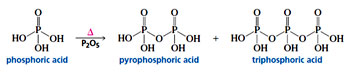
Heating of phosphoric acid molecules results in formation of pyrophosphoric acid, which is a phosphoanhydride. formed by loss of a water molecule. The name pyrophosphate is derived from the the Greek word - pyr, which means “fire.” Thus, pyrophosphoric acid is prepared by “fire”—that is, by heating. In the above said manner, Triphosphoric acid and higher polyphosphoric acids can also be formed, due to the ability of phosphoric acid to form an anhydride.
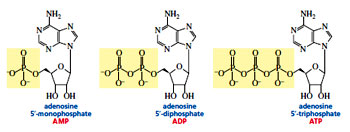
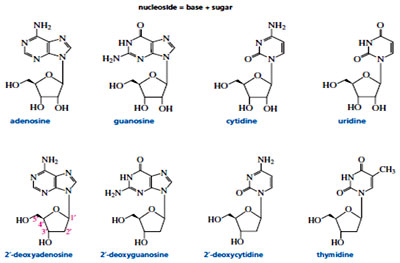
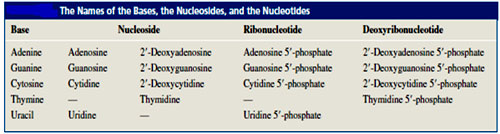
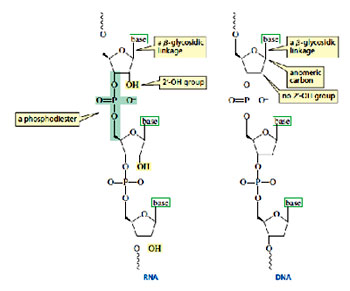
Nucleotides apart from acting as integral units of DNA and RNA, tperform various cellular activities such as donors of chemical energy, secondary messengers in cell signalling, cofactors for enzymes, as vasidialtors, carriers of subunits required in cell wall synthesis and lipid biosynthesis.
ATP- Currency of the cell:
All living systems need energy to ensure their survival and reproduction and they derive energy by converting nutrients into a chemically useful form of energy. The most important form of chemical energy is adenosine -triphosphate (ATP). The importance of ATP to biological reactions is shown by its turnover rate in humans—each day, a person uses an amount of ATP equivalent to his or her body weight. ATP is known as the universal carrier of chemical energy as it is commonly used in all living systems from prokaryotes to eukaryotes. ATP has the ability to convert thermodynamically unfavorable reactions to favorable ones as “the energy of hydrolysis of ATP converts endergonic reactions into exergonic reactions.”
The secret behind the stability of ATP:
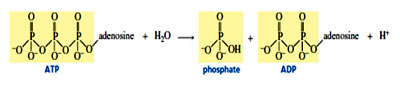
Other Important Nucleotides and teir functions:
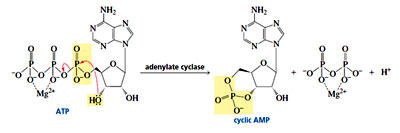
- ATP is not the only biologically important nucleotide. Guanosine –triphosphate (GTP) is also used in place of ATP in some phosphoryl transfer reactions. GTP drives the peptide bond formation during translation. GTP and GDP are also involved in G-protein coupled Receptor (GPCR) mediated signaling.
- UDP serves as the carrier of Glucose moiety in the form of UDP-Glucose, required for cell wall synthesis in bacteria.
- CDP-Choline acts as the carrier of Choline group for the synthesis of membrane phospholipid- Phosphotidyl Choline.
- Dinucleotides such as FAD, FMN are used as oxidizing agents and NADH, NADPH are used as reducing agents.
- Another important nucleotide is adenosine – monophosphate, commonly known as cyclic AMP. Cyclic AMP is called a “second messenger” because it serves as a link between several hormones (the first messengers) and certain enzymes that regulate cellular function. Secretion of certain hormones, such as adrenaline, activates adenylate cyclase, the enzyme responsible for the synthesis of cyclic AMP from ATP. Cyclic AMP then activates an enzyme, generally by phosphorylating it.
- Adenosine acts as Sleep inducer and is commercially available as “Adenocarp”
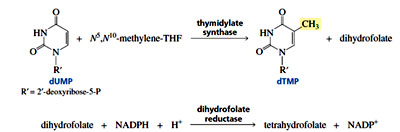
The reducing agent is NADPH and every NADPH produced in the cell can generate three ATPs. Based on the this fact, the usage of NADPH to reduce dihydrofolate comes at the expense of ATP. Although the process of synthesis of thymine is energetically expensive still the cell prefers to recruit Thymine in DNA rather than Uracil.
The presence of uracil in DNA is mutagenic and presence of thymine prevents potentially lethal mutations. The chemical phenomenon behind this is chemical instability of Cytosine which can tautomerize to form an imine, which subsequently gets hydrolyzed to uracil. The overall reaction is called a deamination since it removes an amino group.
If a cytosine in DNA is deaminated to a uracil, uracil will specify incorporation of an adenine into the daughter strand during replication instead of the guanine that would have been specified by cytosine. This would have adverse effects as it leads to changes in the nucleotide sequence and can cause GC basepairs to AT base pair mutations. Inorder to avoid such complications presence of a U in DNA is recognized as a “mistake” by celllular enzymes before an incorrect base can be inserted into the daughter strand. Uracil-N-glycolsylase and dUTPase are the two enzymes which ensure the elimination of U from DNA. dUTPase cleaves UTP molecules present in the nucleus and prevents them for getting into the active site of DNA polymersase. In case of any U being incorporated into the DNA, Uracil-N-glycolsylase cleaves the glycosydic bond and removes the nucleotide frorn DNA. This generates a apyrimidinic site which is recognized as a lesion and is further repaired by repair enzymes.
If U’s were normally found in DNA, the enzymes could not distinguish between a normal U and a U formed by deamination of cytosine. Having T’s in place of U’s in DNA allows the U’s that are found in DNA to be recognized as mistakes. Unlike DNA, which replicates itself, any mistake in RNA does not survive for long because RNA is constantly being degraded and then resynthesized from the DNA template. Therefore, it is not worth spending the extra energy to incorporate T’s into RNA.
Properties of nucleic acids:
Effect of temperature
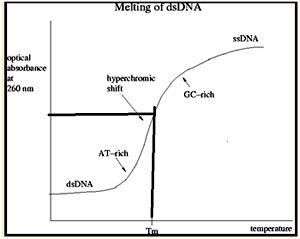
Hyperchromic shift: Increase in the absorption at 260nm upon denaturation of double stranded DNA resulting in formation of single stranded DNA is termed as Hyperchromic shift.
Hypochromic shift: Decrease in the absorption at 260nm upon renaturation of single stranded DNA molecules to yield double stranded DNA is termed as Hypochromic shift. Renaturation bt slow cooling is called Annealing.
The Tm for a given duplex is otherwise affected by a number of factors. The major determinants include sequence length, G-C content and salt concentration. Longer duplexes or those having a higher proportion of G-C pairs are more stable and therefore will have a higher Tm. Monovalent cations, such as sodium (Na+) and potassium (K+), stabilize double helices by partially neutralizing the negative charge along the phosphodiester backbone. Consequently, higher salt concentration will also increase the Tm of a given polynucleotide duplex. In addition the presence of mismatched bases in a duplex will generally have a destabilizing effect and decrease Tm.
Properties that get altered upon Denaturation:
- Absorbance At 260 nm in decreasing order.
Absorbance of free nucleotides is more their polymeric forms and single stranded molecules absorb more than double stranded forms. Similarly absorption of RNA is more than DNA due to the presence of 2’-OH group. Purine absorb more than pyrimidines due to the presence of double ring structure. Amongst the purines, Adenine absorbs more than Guanine due to the presence of more free electrons. Consequently two DNA molecules equal in length but differing in GC content absorb differently with the DNA having more AT content absorbing more than the DNA with more GC content.
A260: Nucleotides > ss DNA > ds DNA
A260: RNA > DNA
A260: Purines > Pyrimidines
A260: Adenine > Guanine > Thymine = Cytosine
- Osmotic pressure increases upon denaturation. Organic solvents and detergents interferes hydrophobic interactions and causes the DNA denaturation.
- Viscosity decreases upon denaturation. DNA solutions are highly viscous and high viscosity is due to the long length and rigidity of the molecules. Upon denaturation, viscosity decreases as double stranded DNA is more rigid and hence viscous when compared to single stranded DNA.
- Optical Rotation becomes more negative upon denaturation as the plane polarized light shifts slightly towards left (more negative).
- Density of nucleic acids increases upon denaturation as double stranded DNA is less dense when compared to single stranded DNA as it occupies more mass per given volume.
Effect of pH on nucleic acids:
Nucleic acids are stable between a pH range of 5-9. Beyond this range, they undergo denaturation spontaneously due to the tautomeric shift of Nitrogen bases altering the H- bonding between the nucleotides of the opposite strands.
Effect of Acid on nucleic acids:
Both DNA and RNA undergo acid hydrolysis. The glycosidic bonds of purine nucleosides are susceptible to acid hydrolysis by protonation at N7 of guanosine or adenosine. Under mild acidic conditions DNA becomes Apurinic DNA. Depurination of DNA is more favourable than that of RNA, although the formation of an abasic site in the polynucleotide results in either case. A complete loss of sequence information represented by an abasic site in DNA can likewise cause a permanent mutation.
Effect of Alkali on nucleic acids:
RNA undergoes alkali hydrolysis due to the presence of 2’-OH group but DNA doesn’t undergo alkali hydrolysis.
Although both RNA and DNA can be degraded through cleavage of the phosphodiester backbone, their chemical stability is drastically different. RNA is distinctively subjected to a transesterification reaction in which the C2’ oxygen serves as a nucleophile for attack on the adjacent phosphorus. This particular reaction proceeds through a pentacoordinate phosphate intermediate, in which the bond formed between the C2’ oxygen and the phosphorus centre which is in line with the bond to be broken between the phosphorus centre and the C5’ oxygen. As a aresult, the reaction products terminate with a C5’ hydroxyl and C2’, C3’-cyclic phosphate. This cyclizing mechanism of RNA transesterification occurs easily because the nucleophile is inherently positioned in proximity to the phosphorus centre in the phosphodiester backbone. Moreover, the reaction is promoted either by deprotonation of the C2’ hydroxyl or protonation of the C5’ oxygen. Therefore, RNAs are easily degraded in alkaline or acidic solutions. A similar mechanism applicable to both RNA and DNA polymers, operates for cleavage of phosphodiester , in which a second molecule serves as the attacking nucleophile. The reaction is similarly promoted by deprotonation of the attacking group and protonation of the leaving group. However, the products terminate with a C5’ phosphate and C3’ hydroxyl. Water can serve as a nucleophile in the spontaneous hydrolytic degradation of RNA or DNA polymers, although the reaction is approximately 100 000 times less likely to occur than RNA transesterification. Based on the above principle and the fact that each sugar lacks a C2’ hydroxyl group, the phosphate and sugar backbone of DNA exhibits a relatively huge chemical stability. In fact, DNA is the most stable of the biological polymers enabling it to maintain the integrity of genetic information- a prerequisite to act as genetic material.
Effect of bisulfite and nitrous acid:
Bisulfite and nitrous acid promote deamination or removal of an amino group from cytosine, resulting in conversion of cytosine to uracil. In DNA, cytosine deamination will ultimately lead to conversion of a G-C base pair to an A-T base pair if left uncorrected.
Effect of Ionizing radiations and Oxidizing agents:
Strong oxidizing agents such as hydroxyl radicals generated by ionizing radiation or incomplete metabolic reduction of oxygen producing superoxide ions, hydrogen peroxide or hydroxyl radicals react extremely with DNA. Hydroxyl radicals can nonspecifically damage DNA by breaking the phosphodiester backbone. Strand scission occurs by a number of mechanisms, each of which produces polynucleotide fragments with different 5’and 3’ terminal products. In addition, hydroxyl radicals can directly attack and alter the chemical composition of the bases in nucleic acids generating compounds like 8-hydroxyguanine and thymine glycol. These modifications result in formation of lesions in the sequence of DNA making them susceptible to mutations, which may ultimately lead to cancer. Therefore, ionizing radiation and other compounds that cause DNA damage are typically carcinogenic or cancer causing.
Forces stabilizing nucleic acid structure:
- Sugar- phosphate chain confirmations:
Sugar-phosphate back bone has 6 torsion angles and N-C1 glycosidic bond also exerts torsion in a nucleotide unit. There angles are subject to variety of internal contrasting forces that restrict this conformational freedom. Glycosidic bonds have only one or two stable positions. The rotation of a base around its glycosidic bond is greatly hindered.
Pyrimidine bases are stable only in anti confirmations as the sugar residues experience stearic hindrance with its pyrimidine substations in syn confirmation, Alternatively, purine nucleotides are stable in both syn and anti confirmations. In double helical nucleic acids, bases are mostly stable in anti-conformation.
- Sugar ring pucker :
The ring substituents are eclipsed when the ring is planar and in order to releive the resultant crowding which even occurs between atoms the ring puckers , it becomes slightly non planar.
Four of five atoms present are co-planar lying close to each other within few hundredths of angstroms and the remaining atoms are out if the plane by several angstroms. If the atom, out of plane is displaced to the same side of the ring as atom C5', it is said to have 'endo' (within), whereas if the displacement is towards the opposite side of the ring from C5', it is known as 'exo' confirmation (out of).C2' endo confirmation is most frequently occurring and C3’exo and C3' endo is also common.
The ribose pucker is confirmationally important in nucleic acid because it governs the relative orientations of the phosphate substituents to each ribose residues.
- Base pairing:
Hoogstein base pairing: A-T base pairing invariables have adenine N7 as the hydrogen bonding acceptor rather than N1. This suggest that Hoogsten bp is inheritantly more sable for A-T pairs than is Watson crick pairing. Stearic and other environmental factors influences make Watson crick geometry the preferred mode of base pairing. Hoogsten bp stabilizes tertiary structure of t RNA.
Base stacking interaction: purines and pyrimidine’s tend to form extended stacks of planar parallel molecules. This has been observed in the several hundred reported X-ray crystal structure that contain nucleic acid. Stacking interaction are a type of vanderwaals interaction in the solid state.
- Hydrophobic forces: Hydrophobic interactions are a type of vanderwaals interactions and are the major stabilizing forces that not only stabilize nucleic acids but also proteins. These interactions in nucleic acid are enthalpically driven and in case of proteins are entropically driven.
- Ionic Interaction: Divalent cations are more effective shielding agents for nucleic acids than are monovalent cations. Polyelectrolytes are more commonly involved in ionic interactions.
Published date : 19 Jun 2014 05:16PM