Cell Strucure- II
Sakshi Education
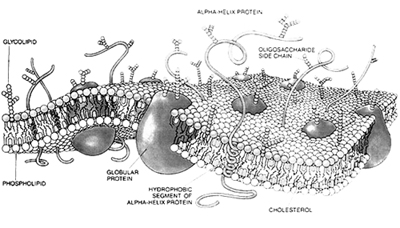
The Cell Surface and Plasma Membrane
The cell surface and the cell (plasma) membrane also differ between eukaryotic and prokaryotic cells. As mentioned earlier, the cell surface of some prokaryotic cells is covered with a double membrane, as well as an external cell wall of cross-linked polysaccharides like N-acetyl Glucoseamine and N-acetyl Muramic acid. A mucous ‘coat’ often also lies around this outer wall.
Among eukaryotic cells, animal cells are characterized by the absence of cell wall and in fact have been the primary basis for categorizing them into a different group or kingdom under different classification system. Instead, they possess a glycocalyx of carbohydrate-rich transmembrane molecules that straddle the outer plasma membrane and enable adjacent cells to stick to one another provided they are present at a limiting distance of 15–20 nm. This spacing permits the recognition, by circulating chemical signals, of membrane-associated cellular receptor or adhesion molecules. However plants posses an external cell wall, which is primarily composed of cellulose fibrils to give strength and rigidity.
The plasma membrane is a lipid bilayer interspersed by a number of proteins, arranged in a variety of patterns that are related to their function. Plasma membrane has been described as a sea of lipid bilayer in which proteins are like ‘icebergs’ which have the capacity to move translaterally. The membrane is therefore said to have a ‘fluid’ character.
Various models have been proposed over the years to explain its molecular structure. The first model explaining the membrane structure was devised by Davson and Danielli called the Davson–Danielli "Lipid Bilayer Model". J. David Robertson proposed a new model which he called the ‘unit’ membrane model; stating that all membranes shared a single, unifying structure. The most accepted ‘fluid-mosaic’ model was proposed by Singer and Nicolson in 1982. The word ‘mosaic’ reflects the fact that various micro-domains consisting of proteins in membrane, even from different parts of the same eukaryotic cell, may have different patterns, as is found in the mosaic floors of ancient Roman villas.
The bilayer structure of the membrane permits the lateral motion of lipids and proteins, but not normally flipflop movement, i.e. shifting from inside to outside or vice versa; this can occur only rarely, in the case of lipids with the aid of ‘flippase’ enzymes.
The membrane therefore is asymmetrical, with certain proteins facing the extracellular space or the outside environment, and others the internal cytoplasm to meet the various requirements of the cell. For example, receptor protein molecules that can be recognized by circulating hormones, antibodies or other molecules faces towards outside, whereas some other involved in second messenger signalling system might face towards cytoplasm. On the other hand proteins acting as ‘Channels’ are found straddling the plasma membrane allowing the inward or outward passage of ions. Thus proteins play different roles in recognition or anchoring, and may be peripheral or extrinsic, as distinct from integral or intrinsic, to the lipid bilayer. The anchors observed at the cytoplasmic surface tend to be the cytoskeletal elements, the microfilaments, intermediate filaments or the microtubules, as well as G proteins.
The external face has projecting carbohydrate moieties, which produce the ‘glycocalyx’ of the plasma membrane.
All cells contain the plasma membrane which separates the interior cytoplasm of the cell from the external environment. Similar architecture of the membranes is also observed in the membranes internal to the cell leading to the compartmentalization.
Internal membranes partition the cytoplasm into separate compartments concentrating specific substrates and enzymes for particular cellular activities to execute different cellular functions. Since the cytoplasm surrounding the nucleus in the cell is called the cell body, these diminutive compartments have been termed ‘organelles’.
Endoplasmic reticulum
One of the most striking cellular organelles is the endoplasmic reticulum (ER), composed of numerous flattened membranous cisternae lying throughout the cytoplasm; this is in continuity with the double nuclear envelope. It is often studded with ribosomes; if so, it is termed rough endoplasmic reticulum (RER); the RER makes proteins for export. If ribosomes are absent from the cisternae, it is called smooth endoplasmic reticulum (SER); SER is involved in lipid or steroid synthesis.
Transfer RNA (tRNA) is also required during the process of protein synthesis, to bring the subunits of proteins, amino acids, into a linear array that is coded for by the mRNA. The ribosomal RNA (rRNA) is contained within the ribosomes, which act as the factory or sites in which the requisite components assemble together to synthesize the linear protein molecule. This process is termed translation and takes place in the cytoplasm.
In eukaryotic cells this new protein molecule subsequently folds up within the cisternae of the RER, into which it has been inserted during the process of its synthesis by the ribosomes, to form the mature, functional form of the protein, destined for export.
The protein enters the ER cisternae by a process commonly referred to as the ‘signal hypothesis’, whereby a terminal signal sequence of the protein being synthesized tells the cell that the protein should be inserted, via a pore through the RER membrane, into the ER cisternal space there.
The linear protein finally folds up, inside the ER cisternae, into a three-dimensional functional form that is maintained, mainly by weak secondary forces, in the most thermodynamically stable configuration. Proteins can also be synthesized for use by the cell, without entering the ER cisternae at all. This is always the case in prokaryotic cells, which lack ER.
Endosomes
Golgi Apparatus
The structure was first described as a network or reticulum by Camillo Golgi, in nerve cells which were later referred to as Golgi’s bodies, Golgi complexes or the Golgi apparatus. Golgi Apparatus is a series of stacked saccules associated with vesicles and granules. These stacks of saucers exhibit with two distinct faces, a ‘forming’ and a ‘maturing’ face. Proteins transported from the RER are received by the forming face and perform essential modifications like glycosylation (O-linked glycosylation) and further condense them into dense granules called Secretory granules. These granules are dispatched from the maturing face by a budding (pinching off) process.
The secretory products are destined to be released outside the cell and thus granules translocate towards the membrane with the aid of cytoskeletal proteins like microtubules and fuse with the plasma membrane becoming an integral part of it subsequent to secretion of the products. This process of secretion is termed as Exocytosis.
In the same manner any exogenous material can be brought into the cell by the process of Endocytosis. Solid material if brought in by Endocytosis, it is called Phagocytosis and internalization of liquid material is called Pinocytosis. Endocytosis is marked by the formation of special pits embedded by a protein called Cathrin, around the material to be brought into the cell. Cathrin coated pits bud off internally from the plasma membrane to fuse with the internal membrane leading to formation of Endosome.
Lysosomes
Lysosomes are synthesized from the maturing face of Golgi the area being referred as GERL. The area is concentrated with different enzymes especially lyric enzymes which are loaded into then forming primary lysosomes.The endosomes are moved to the Golgi area, where their contents may be utilized or degraded by lysosomes.
Once a primary lysosome has fused with an endosome and starts degrading its contents, it becomes a secondary lysosome. Lysosomes have been called ‘suicide bags’ because their enzymatic contents capable of degrading biomolecules. The pH within the lysosome is about pH 5, substantially less than that of the cytosol (~pH 7.2) which helps in the degradation process as most of the lysosomal enzymes work better at the pH. The products of the catabolic breakdown of the endosomal contents move out, through the lysosomes’ peripheral semipermeable membrane, into the ground cytoplasm to be reutilized. This is the reason why some organelles like lysosomes are single membraned so as to facilitate the easy diffusion of molecules.In the event of the contents being non degradable, they accumulate and the lysosomes are termed differently as Residual bodies which are secreted by the Golgi.
Peroxisomes
Peroxisomes are nearly similar to lysosomes in being single membrane. A major function of the peroxisome is the breakdown of very long chain fatty acids through beta-oxidation and degradation of hydrogen peroxide, but differ drastically due to the presence of a special spectrum of enzymes – d-amino acid oxidase, catalase and peroxidase. By using molecular oxygen, hydrogen atoms are removed from specific organic substrates (labeled as R), in an oxidative reaction, producing hydrogen peroxide (H2O2, a toxic byproduct of cellular metabolism):
RH2 + O2 ? R + H2O2
Catalase uses H2O2 generated by other enzymes in the peroxisome to oxidize other substrates, including phenols, formic acid, formaldehyde and alcohol, by means of the peroxidation reaction:
H2O2 + R'H2 ? R' + 2H2O
This reaction is important in liver and kidney cells where the peroxisomes detoxifiy various toxic substances that enter the blood. About 25% of the ethanol consumed is oxidized to acetaldehyde in this way. In addition, when excess H2O2 accumulates in the cell, catalase converts it to H2O through this reaction:
2H2O2 ? 2H2O + O2
A major function of the peroxisome is the breakdown of fatty acid molecules, in a process called beta-oxidation. In this process, the fatty acids are broken down two carbons at a time, converted to Acetyl-CoA, which is then transported back to the cytosol for further use. In animal cells, beta-oxidation can also occur in the mitochondria. In yeast and plant cells this process is exclusive for the peroxisome.
The first reactions in the formation of plasmalogen in animal cells also occurs in peroxisomes. Plasmalogen is the most abundant phospholipid in myelin. Deficiency of plasmalogens causes profound abnormalities in the myelination of nerve cells, which is one of the reasons that many peroxisomal disorders lead to neurological disease. Peroxisomes also play a role in the production of bile acids.
A deficiency in the protein import can lead to empty peroxisomes, leading to abnormalities in the brain, called Zellweger syndrome.
Vacuoles
Vacuoles are membrane-bounded compartments within some eukaryotic cells that can serve a variety of secretory, excretory, and storage functions. Vacuoles and their contents are considered to be distinct from the cytoplasm, and are classified as ergastic according to some authors (Esau, 1965). Vacuoles are especially conspicuous in most plant cells. Most mature plant cells have a single central vacuole which often takes up more than 80% of the cell interior. It is surrounded by a membrane called the tonoplast.
In general, vacuole functions include:
Mitochondra
Mitochondria are about 2–3 mm a bit larger than the lysosomes Mitochondria are regarded as the “Power houses of the cell" and are concerned with cellular respiration. They possess both the components of the Krebs (or tricarboxylic acid) cycle and the proton pumps and ATP synthase and thus generate adenosine triphosphate (ATP), the source of cellular energy. Mitochondria are double membraned where the inner membrane is thrown into a series of folds cristae.
These cristae are studded with ‘stalked particles’, the stalk of which is called F0 and the head F1. The F0 is the proton channel across the inner mitochondrial membrane through which the hydrogen ions (protons) flow. This flow triggers the ATP synthase present in the F1 head to generate ATP. According to endosymbiontic theory mitochondria has prokaryotic origin, settled in eukaryotic cells.Support for this contention comes from the presence of 70S ribosomes and circular, naked DNA, similar to prokaryotic cells, within their matrix, or inner space. Their DNA codes for some, but not all, of their own component proteins, so mitochondria are only semiautonomous.
Chloroplasts are organelles that are found only in the cells of plants and are the basis of photosynthesis. Similar to mitochondria they too have two outer membranes but are rather larger. Additionally a third set of folded internal membranes is also present which make up the thylakoids, or stacked, chlorophyll-rich lamellae. The internal space of the chloroplast is called Stroma where the Calvin pathway, or ‘dark’ reaction occurs. in the storms or . This is the pathway by which sugars are made from carbon dioxide and water.
Like the mitochondria, these organelles also possess circular DNA and 70S ribosomes in their stromal space, and are thought to have evolved from symbiotic photosynthetic prokaryotes.
The ‘light’ reaction occurs in the thylakoid membranes, whereby photosystems I and II of the chlorophyll reactive centres capture the energy of sunlight and harness it to the production of reduced coenzymes and a proton gradient. Protons flowing down the gradient lead to the production of the energy-rich ATP via CF0 (a proton channel) and CF1 (ATP synthase) in the chloroplast thylakoid membrane.
Microtubules, as the name suggests, are tiny tubules, 23– 25nm in diameter. Their basic subunit is a dumbbell shaped heterodimer of a- and b-tubulin. These polymerize into protofilaments and there are usually 13 of these forming the wall of the microtubule around a hollow core. They not only comprise the spindle, but also from the 912 arrays in the motile cilia and flagella, and are the cables along which proteins are transported from the nerve cell body of neurons to the terminal synapse, through the cytoplasmic axonal process.
They appear to be associated with ‘motor’ molecules such as dynein, kinesin or myosin, which empower the transport of, for example, vesicles along the microtubules of the axonal process.Similarly, microfilaments are constructed of subunits of globular actin, called G-actin, which are linked into polymers called filamentous, or F-actin. This is the functional form which makes up the microfilaments, 4–6 nm in diameter, which lie as twin cables, intertwining in a helical array.
These act as the devices to maintain the shape of cellular projections such as the microvilli of intestinal cells (Figure 2), which increase the cellular surface area dramatically in a highly advantageous way for absorption of nutritional molecules. They are also involved in cellular motility and, in muscle fibres, make up a major subcomponent, actin, which lies alongside myosin, the motor molecule. In striated, or skeletal, muscle, as well as cardiac muscle, this is a highly ordered, close-packed array of the two molecules; this packing is less regular in smooth muscle.
The two sets of filaments, actin and myosin, slide past each other during muscle contraction, the myosin heads ‘walking’along the actin fibrils. This activity requires ATP as well as calcium (Ca21) ions; the latter are stored in cisternae of ER, ready for instant release when a muscular contraction is required. The Ca21binds to another molecule, troponin, which shifts tropomyosin molecules, which in turn allow the myosin heads to interact with the actin, thereby activating the sliding filament motion.
Without ATP, the muscle filaments cannot slide past each other, as the myosin heads cannot release and reattach to the actin, and ‘rigor mortis’ ensues. The intermediate filaments may differ chemically in different cell types but they all share certain similar structural characteristics. They are composed of varying numbers of fibrous subunits, which associate side by side into rope-like structures that possess enormous tensile strength. They are found around the inner nuclear envelope, as so-called ‘nuclear lamins’, as well as throughout the cell cytoplasm, anchoring on to the plasma membrane at specific junctures, the spot desmosomes, thereby acting as joints or junctions to hold adjacent cells together. These filaments can accumulate as keratin in dead cells, persisting to form the hair and nails.
Other subnuclear structures:
Besides the nucleolus, the nucleus contains a number of other non-membrane delineated bodies.
Cajal bodies: A nucleus will contain between 1 and 10 compact structures called Cajal bodies. Their diameter measures between 0.2µm and 2.0µm depending on the cell type and species, and when seen under an electron microscope, appear as balls of tangled thread. They are involved in a number of different roles relating to RNA processing, specifically snoRNA and snRNA maturation, and histone mRNA modification.
PML bodies: These are spherical bodies found scattered throughout the nucleoplasm, measuring around 0.2-1.0µm. They are known by a number of other names, including nuclear domain 10 (ND10), Kremer bodies, and PML oncogenic domains. They are often seen in the nucleus in association with Cajal bodies and cleavage bodies. It has been suggested that they play a role in regulating transcription.
Others: Polymorphic interphase karyosomal association (PIKA), Gemini of coiled bodies (GEMS) and Interchromatin granule clusters, also called "speckles".
The cell nucleus contains the majority of the cell's genetic material, in the form of DNA molecules.
Multicellularity
Prokaryotic cells are always unicellular, but eukaryotic cells are the subunits of all multicellular organisms – plants and animals. These cells are able to form multicellular systems because of their capacity to become differentiated or specialized. This is possible because of their compartmentalization into organelles. Cells become specialized for different tasks depending on the arrangement of their internal compartments.
The differentiated state requires a reorganization or redistribution of certain of the major organelles, which will be different for each kind of specialized cell. Muscle cells require much energy, so have many ATP-generating mitochondria, while secretory cells, such as the pancreas, have extensive Golgi bodies, to help produce the requisite secretory products. This division of labour means that certain organs, made up of a certain kind of specialized cell, can be specially programmed for a particular function. The brain serves as a good example, as it is a collection of highly differentiated neurons or nerve cells (surrounded by supportive glial cells), which synthesize and exchange chemical signals that permit nervous functioning, such as the capacity of animals to respond to their environment.
Intercellular Junctions: Communication and Adhesion
Cells in a multicellular organism are linked together within organs by a variety of different intercellular junctions. These include: (1) adhering (adherens) junctions, such as the desmosomes, intermediate junctions and septate junctions (unique to the tissues of invertebrates); (2) the occluding junctions, such as the tight junctions; and (3) the communicating junctions, such as the gap junctions, which couple cells together. These are all variations on a theme of plasma membrane modification and serve to maintain the integrity of tissues by holding adjacent cells together. Desmosomes are associated with underlying intermediate filaments, and the intermediate and tight junctions with actin microfilaments (Figure 2). Septate junctions, characterized by ladder-like septa straddling the intercellular cleft, seem to have associations with both actin and microtubules.
Gap junctions appear to have no internal cytoskeletal attachments but they are responsible for cell–cell communication, rather than merely holding adjacent cells together. They are formed by transmembrane subunits, connexons, which become precisely aligned in adjacent cells. Each connexon has a central pore, or channel, which is spatially associated with one of the adjacent cell’s connexons, so that ions and small molecules can be exchanged between cells via the channels. This allows the cells to communicate, as they can pass regulatory molecular morphogens from one cell to the other. The cells are then said to be ‘coupled’. They can become ‘uncoupled’ by conditions such as high pH or calcium concentration, which cause the channels to become closed down. This appears to occur by a configurational change in the six hexameric subunits that make up each connexon. Plant cells, which possess thick cellulose cell walls, can only exchange information via plasmodesmata, which run between the cytoplasm of adjacent cells. These are tiny cytoplasmic passageways through the cellulose walls that carry a minute strand of ER from one cell to the next, through which molecules may move.
Differentiation
All new individuals arise from fertilized eggs, or zygotes, which then divide many times thereafter to produce embryos, then adults. The egg cell cytoplasm contains an organized system of determinants which assign cells to different developmental pathways. These cytoplasmic differences require the zygote nuclear gene expression. This selective activation and expression of specific genes leads to cell and tissue differentiation. The details of the embryonic pattern are filled in by mechanisms which sort cells into particular pathways of differentiation by their relative position in the developing organism.
Groups of cells generate positional information, perhaps due to gradients of diffusible substances within boundaries in tissues, leading to ‘pattern’ formation that is seen, for example, in limb formation. Genetic studies have led to the discovery of homeotic (‘HOX’) genes (master regulatory genes), which determine the mainstream developmental pathways of the different appendages (e.g. arms versus legs) or units of the body plan of organisms. Developmental cell biology is rapidly emerging as the branch of the subject producing some of the most exciting revelations about the way the complex pattern of differentiated tissues becomes organized.
Communication
The developing multicellular organism becomes divided into groups of cells cooperating as tissues. These must selectively adhere to one another to remain as organs, and their component cells must also be able to communicate with each other if division of labour is to occur effectively. This they do by a number of devices, of which one of the most important is the nervous system, where signals are sent by chemical neurotransmitters from one neuron to other cells. Hormones, protein or steroid, also have profound chemical effects on developmental processes, including the acquisition of secondary sexual characteristics, by activating different genes in the DNA. Circulating hormones may also trigger secondary signalling pathways. Local hormones play important roles in adjacent cellular activity, as may other chemicals that operate by chemotaxis or via chemical attractants. Signalling via cell surface molecules also occurs in the immune system, which is based on cellular signals and responses that activate special B and T cells in the lymphoid system, using either humoral or cellmediated mechanisms.
This is important in enabling the organism to survive infection. Growth factors and cellular adhesion molecules may also affect the patterns of differentiation of cells in the formation, and then the maintenance, of organs. This may involve the assembly of the cell–cell junctions, described earlier, which keep the adjacent cells associated together in particular arrangements.
The interplay of chemical signals between the cells of the same or different organs produces an orchestration of cellular activity resulting in a successful, functional organism. The healthy organism, therefore, is dependent on the effective functioning of the cells that make up its organs.
Further Reading
The cell surface and the cell (plasma) membrane also differ between eukaryotic and prokaryotic cells. As mentioned earlier, the cell surface of some prokaryotic cells is covered with a double membrane, as well as an external cell wall of cross-linked polysaccharides like N-acetyl Glucoseamine and N-acetyl Muramic acid. A mucous ‘coat’ often also lies around this outer wall.
Among eukaryotic cells, animal cells are characterized by the absence of cell wall and in fact have been the primary basis for categorizing them into a different group or kingdom under different classification system. Instead, they possess a glycocalyx of carbohydrate-rich transmembrane molecules that straddle the outer plasma membrane and enable adjacent cells to stick to one another provided they are present at a limiting distance of 15–20 nm. This spacing permits the recognition, by circulating chemical signals, of membrane-associated cellular receptor or adhesion molecules. However plants posses an external cell wall, which is primarily composed of cellulose fibrils to give strength and rigidity.
The plasma membrane is a lipid bilayer interspersed by a number of proteins, arranged in a variety of patterns that are related to their function. Plasma membrane has been described as a sea of lipid bilayer in which proteins are like ‘icebergs’ which have the capacity to move translaterally. The membrane is therefore said to have a ‘fluid’ character.
Various models have been proposed over the years to explain its molecular structure. The first model explaining the membrane structure was devised by Davson and Danielli called the Davson–Danielli "Lipid Bilayer Model". J. David Robertson proposed a new model which he called the ‘unit’ membrane model; stating that all membranes shared a single, unifying structure. The most accepted ‘fluid-mosaic’ model was proposed by Singer and Nicolson in 1982. The word ‘mosaic’ reflects the fact that various micro-domains consisting of proteins in membrane, even from different parts of the same eukaryotic cell, may have different patterns, as is found in the mosaic floors of ancient Roman villas.
The bilayer structure of the membrane permits the lateral motion of lipids and proteins, but not normally flipflop movement, i.e. shifting from inside to outside or vice versa; this can occur only rarely, in the case of lipids with the aid of ‘flippase’ enzymes.
The membrane therefore is asymmetrical, with certain proteins facing the extracellular space or the outside environment, and others the internal cytoplasm to meet the various requirements of the cell. For example, receptor protein molecules that can be recognized by circulating hormones, antibodies or other molecules faces towards outside, whereas some other involved in second messenger signalling system might face towards cytoplasm. On the other hand proteins acting as ‘Channels’ are found straddling the plasma membrane allowing the inward or outward passage of ions. Thus proteins play different roles in recognition or anchoring, and may be peripheral or extrinsic, as distinct from integral or intrinsic, to the lipid bilayer. The anchors observed at the cytoplasmic surface tend to be the cytoskeletal elements, the microfilaments, intermediate filaments or the microtubules, as well as G proteins.
The external face has projecting carbohydrate moieties, which produce the ‘glycocalyx’ of the plasma membrane.
Internal Membranes and Subcellular Compartments:
All cells contain the plasma membrane which separates the interior cytoplasm of the cell from the external environment. Similar architecture of the membranes is also observed in the membranes internal to the cell leading to the compartmentalization.
Internal membranes partition the cytoplasm into separate compartments concentrating specific substrates and enzymes for particular cellular activities to execute different cellular functions. Since the cytoplasm surrounding the nucleus in the cell is called the cell body, these diminutive compartments have been termed ‘organelles’.
Endoplasmic reticulum
One of the most striking cellular organelles is the endoplasmic reticulum (ER), composed of numerous flattened membranous cisternae lying throughout the cytoplasm; this is in continuity with the double nuclear envelope. It is often studded with ribosomes; if so, it is termed rough endoplasmic reticulum (RER); the RER makes proteins for export. If ribosomes are absent from the cisternae, it is called smooth endoplasmic reticulum (SER); SER is involved in lipid or steroid synthesis.
Transfer RNA (tRNA) is also required during the process of protein synthesis, to bring the subunits of proteins, amino acids, into a linear array that is coded for by the mRNA. The ribosomal RNA (rRNA) is contained within the ribosomes, which act as the factory or sites in which the requisite components assemble together to synthesize the linear protein molecule. This process is termed translation and takes place in the cytoplasm.
In eukaryotic cells this new protein molecule subsequently folds up within the cisternae of the RER, into which it has been inserted during the process of its synthesis by the ribosomes, to form the mature, functional form of the protein, destined for export.
The protein enters the ER cisternae by a process commonly referred to as the ‘signal hypothesis’, whereby a terminal signal sequence of the protein being synthesized tells the cell that the protein should be inserted, via a pore through the RER membrane, into the ER cisternal space there.
The linear protein finally folds up, inside the ER cisternae, into a three-dimensional functional form that is maintained, mainly by weak secondary forces, in the most thermodynamically stable configuration. Proteins can also be synthesized for use by the cell, without entering the ER cisternae at all. This is always the case in prokaryotic cells, which lack ER.
Endosomes
Golgi Apparatus
The structure was first described as a network or reticulum by Camillo Golgi, in nerve cells which were later referred to as Golgi’s bodies, Golgi complexes or the Golgi apparatus. Golgi Apparatus is a series of stacked saccules associated with vesicles and granules. These stacks of saucers exhibit with two distinct faces, a ‘forming’ and a ‘maturing’ face. Proteins transported from the RER are received by the forming face and perform essential modifications like glycosylation (O-linked glycosylation) and further condense them into dense granules called Secretory granules. These granules are dispatched from the maturing face by a budding (pinching off) process.
The secretory products are destined to be released outside the cell and thus granules translocate towards the membrane with the aid of cytoskeletal proteins like microtubules and fuse with the plasma membrane becoming an integral part of it subsequent to secretion of the products. This process of secretion is termed as Exocytosis.
In the same manner any exogenous material can be brought into the cell by the process of Endocytosis. Solid material if brought in by Endocytosis, it is called Phagocytosis and internalization of liquid material is called Pinocytosis. Endocytosis is marked by the formation of special pits embedded by a protein called Cathrin, around the material to be brought into the cell. Cathrin coated pits bud off internally from the plasma membrane to fuse with the internal membrane leading to formation of Endosome.
Lysosomes
Lysosomes are synthesized from the maturing face of Golgi the area being referred as GERL. The area is concentrated with different enzymes especially lyric enzymes which are loaded into then forming primary lysosomes.The endosomes are moved to the Golgi area, where their contents may be utilized or degraded by lysosomes.
Once a primary lysosome has fused with an endosome and starts degrading its contents, it becomes a secondary lysosome. Lysosomes have been called ‘suicide bags’ because their enzymatic contents capable of degrading biomolecules. The pH within the lysosome is about pH 5, substantially less than that of the cytosol (~pH 7.2) which helps in the degradation process as most of the lysosomal enzymes work better at the pH. The products of the catabolic breakdown of the endosomal contents move out, through the lysosomes’ peripheral semipermeable membrane, into the ground cytoplasm to be reutilized. This is the reason why some organelles like lysosomes are single membraned so as to facilitate the easy diffusion of molecules.In the event of the contents being non degradable, they accumulate and the lysosomes are termed differently as Residual bodies which are secreted by the Golgi.
Peroxisomes
Peroxisomes are nearly similar to lysosomes in being single membrane. A major function of the peroxisome is the breakdown of very long chain fatty acids through beta-oxidation and degradation of hydrogen peroxide, but differ drastically due to the presence of a special spectrum of enzymes – d-amino acid oxidase, catalase and peroxidase. By using molecular oxygen, hydrogen atoms are removed from specific organic substrates (labeled as R), in an oxidative reaction, producing hydrogen peroxide (H2O2, a toxic byproduct of cellular metabolism):
RH2 + O2 ? R + H2O2
Catalase uses H2O2 generated by other enzymes in the peroxisome to oxidize other substrates, including phenols, formic acid, formaldehyde and alcohol, by means of the peroxidation reaction:
H2O2 + R'H2 ? R' + 2H2O
This reaction is important in liver and kidney cells where the peroxisomes detoxifiy various toxic substances that enter the blood. About 25% of the ethanol consumed is oxidized to acetaldehyde in this way. In addition, when excess H2O2 accumulates in the cell, catalase converts it to H2O through this reaction:
2H2O2 ? 2H2O + O2
A major function of the peroxisome is the breakdown of fatty acid molecules, in a process called beta-oxidation. In this process, the fatty acids are broken down two carbons at a time, converted to Acetyl-CoA, which is then transported back to the cytosol for further use. In animal cells, beta-oxidation can also occur in the mitochondria. In yeast and plant cells this process is exclusive for the peroxisome.
The first reactions in the formation of plasmalogen in animal cells also occurs in peroxisomes. Plasmalogen is the most abundant phospholipid in myelin. Deficiency of plasmalogens causes profound abnormalities in the myelination of nerve cells, which is one of the reasons that many peroxisomal disorders lead to neurological disease. Peroxisomes also play a role in the production of bile acids.
A deficiency in the protein import can lead to empty peroxisomes, leading to abnormalities in the brain, called Zellweger syndrome.
Vacuoles
Vacuoles are membrane-bounded compartments within some eukaryotic cells that can serve a variety of secretory, excretory, and storage functions. Vacuoles and their contents are considered to be distinct from the cytoplasm, and are classified as ergastic according to some authors (Esau, 1965). Vacuoles are especially conspicuous in most plant cells. Most mature plant cells have a single central vacuole which often takes up more than 80% of the cell interior. It is surrounded by a membrane called the tonoplast.
In general, vacuole functions include:
- Capturing food materials
- Removing unwanted structural debris surrounding the cell
- Sequestering materials that might be toxic to the cell
- Containment of waste products
- Maintaining internal hydrostatic pressure or turgor within the cell
- Maintaining an acidic internal pH
- Storing small molecules
- Exporting unwanted substances from the cell.
- Enabling the cell to elongate rapidly or otherwise alter relative cell size.
Mitochondra
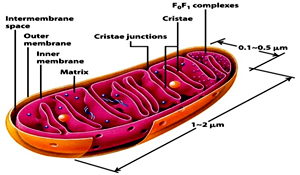
These cristae are studded with ‘stalked particles’, the stalk of which is called F0 and the head F1. The F0 is the proton channel across the inner mitochondrial membrane through which the hydrogen ions (protons) flow. This flow triggers the ATP synthase present in the F1 head to generate ATP. According to endosymbiontic theory mitochondria has prokaryotic origin, settled in eukaryotic cells.Support for this contention comes from the presence of 70S ribosomes and circular, naked DNA, similar to prokaryotic cells, within their matrix, or inner space. Their DNA codes for some, but not all, of their own component proteins, so mitochondria are only semiautonomous.
Chloroplasts
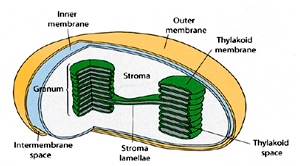
Like the mitochondria, these organelles also possess circular DNA and 70S ribosomes in their stromal space, and are thought to have evolved from symbiotic photosynthetic prokaryotes.
The ‘light’ reaction occurs in the thylakoid membranes, whereby photosystems I and II of the chlorophyll reactive centres capture the energy of sunlight and harness it to the production of reduced coenzymes and a proton gradient. Protons flowing down the gradient lead to the production of the energy-rich ATP via CF0 (a proton channel) and CF1 (ATP synthase) in the chloroplast thylakoid membrane.
Cytoskeleton
Microtubules, as the name suggests, are tiny tubules, 23– 25nm in diameter. Their basic subunit is a dumbbell shaped heterodimer of a- and b-tubulin. These polymerize into protofilaments and there are usually 13 of these forming the wall of the microtubule around a hollow core. They not only comprise the spindle, but also from the 912 arrays in the motile cilia and flagella, and are the cables along which proteins are transported from the nerve cell body of neurons to the terminal synapse, through the cytoplasmic axonal process.
They appear to be associated with ‘motor’ molecules such as dynein, kinesin or myosin, which empower the transport of, for example, vesicles along the microtubules of the axonal process.Similarly, microfilaments are constructed of subunits of globular actin, called G-actin, which are linked into polymers called filamentous, or F-actin. This is the functional form which makes up the microfilaments, 4–6 nm in diameter, which lie as twin cables, intertwining in a helical array.
These act as the devices to maintain the shape of cellular projections such as the microvilli of intestinal cells (Figure 2), which increase the cellular surface area dramatically in a highly advantageous way for absorption of nutritional molecules. They are also involved in cellular motility and, in muscle fibres, make up a major subcomponent, actin, which lies alongside myosin, the motor molecule. In striated, or skeletal, muscle, as well as cardiac muscle, this is a highly ordered, close-packed array of the two molecules; this packing is less regular in smooth muscle.
The two sets of filaments, actin and myosin, slide past each other during muscle contraction, the myosin heads ‘walking’along the actin fibrils. This activity requires ATP as well as calcium (Ca21) ions; the latter are stored in cisternae of ER, ready for instant release when a muscular contraction is required. The Ca21binds to another molecule, troponin, which shifts tropomyosin molecules, which in turn allow the myosin heads to interact with the actin, thereby activating the sliding filament motion.
Without ATP, the muscle filaments cannot slide past each other, as the myosin heads cannot release and reattach to the actin, and ‘rigor mortis’ ensues. The intermediate filaments may differ chemically in different cell types but they all share certain similar structural characteristics. They are composed of varying numbers of fibrous subunits, which associate side by side into rope-like structures that possess enormous tensile strength. They are found around the inner nuclear envelope, as so-called ‘nuclear lamins’, as well as throughout the cell cytoplasm, anchoring on to the plasma membrane at specific junctures, the spot desmosomes, thereby acting as joints or junctions to hold adjacent cells together. These filaments can accumulate as keratin in dead cells, persisting to form the hair and nails.
Other subnuclear structures:
Besides the nucleolus, the nucleus contains a number of other non-membrane delineated bodies.
Cajal bodies: A nucleus will contain between 1 and 10 compact structures called Cajal bodies. Their diameter measures between 0.2µm and 2.0µm depending on the cell type and species, and when seen under an electron microscope, appear as balls of tangled thread. They are involved in a number of different roles relating to RNA processing, specifically snoRNA and snRNA maturation, and histone mRNA modification.
PML bodies: These are spherical bodies found scattered throughout the nucleoplasm, measuring around 0.2-1.0µm. They are known by a number of other names, including nuclear domain 10 (ND10), Kremer bodies, and PML oncogenic domains. They are often seen in the nucleus in association with Cajal bodies and cleavage bodies. It has been suggested that they play a role in regulating transcription.
Others: Polymorphic interphase karyosomal association (PIKA), Gemini of coiled bodies (GEMS) and Interchromatin granule clusters, also called "speckles".
The cell nucleus contains the majority of the cell's genetic material, in the form of DNA molecules.
Multicellularity
Prokaryotic cells are always unicellular, but eukaryotic cells are the subunits of all multicellular organisms – plants and animals. These cells are able to form multicellular systems because of their capacity to become differentiated or specialized. This is possible because of their compartmentalization into organelles. Cells become specialized for different tasks depending on the arrangement of their internal compartments.
The differentiated state requires a reorganization or redistribution of certain of the major organelles, which will be different for each kind of specialized cell. Muscle cells require much energy, so have many ATP-generating mitochondria, while secretory cells, such as the pancreas, have extensive Golgi bodies, to help produce the requisite secretory products. This division of labour means that certain organs, made up of a certain kind of specialized cell, can be specially programmed for a particular function. The brain serves as a good example, as it is a collection of highly differentiated neurons or nerve cells (surrounded by supportive glial cells), which synthesize and exchange chemical signals that permit nervous functioning, such as the capacity of animals to respond to their environment.
Intercellular Junctions: Communication and Adhesion
Cells in a multicellular organism are linked together within organs by a variety of different intercellular junctions. These include: (1) adhering (adherens) junctions, such as the desmosomes, intermediate junctions and septate junctions (unique to the tissues of invertebrates); (2) the occluding junctions, such as the tight junctions; and (3) the communicating junctions, such as the gap junctions, which couple cells together. These are all variations on a theme of plasma membrane modification and serve to maintain the integrity of tissues by holding adjacent cells together. Desmosomes are associated with underlying intermediate filaments, and the intermediate and tight junctions with actin microfilaments (Figure 2). Septate junctions, characterized by ladder-like septa straddling the intercellular cleft, seem to have associations with both actin and microtubules.
Gap junctions appear to have no internal cytoskeletal attachments but they are responsible for cell–cell communication, rather than merely holding adjacent cells together. They are formed by transmembrane subunits, connexons, which become precisely aligned in adjacent cells. Each connexon has a central pore, or channel, which is spatially associated with one of the adjacent cell’s connexons, so that ions and small molecules can be exchanged between cells via the channels. This allows the cells to communicate, as they can pass regulatory molecular morphogens from one cell to the other. The cells are then said to be ‘coupled’. They can become ‘uncoupled’ by conditions such as high pH or calcium concentration, which cause the channels to become closed down. This appears to occur by a configurational change in the six hexameric subunits that make up each connexon. Plant cells, which possess thick cellulose cell walls, can only exchange information via plasmodesmata, which run between the cytoplasm of adjacent cells. These are tiny cytoplasmic passageways through the cellulose walls that carry a minute strand of ER from one cell to the next, through which molecules may move.
Differentiation
All new individuals arise from fertilized eggs, or zygotes, which then divide many times thereafter to produce embryos, then adults. The egg cell cytoplasm contains an organized system of determinants which assign cells to different developmental pathways. These cytoplasmic differences require the zygote nuclear gene expression. This selective activation and expression of specific genes leads to cell and tissue differentiation. The details of the embryonic pattern are filled in by mechanisms which sort cells into particular pathways of differentiation by their relative position in the developing organism.
Groups of cells generate positional information, perhaps due to gradients of diffusible substances within boundaries in tissues, leading to ‘pattern’ formation that is seen, for example, in limb formation. Genetic studies have led to the discovery of homeotic (‘HOX’) genes (master regulatory genes), which determine the mainstream developmental pathways of the different appendages (e.g. arms versus legs) or units of the body plan of organisms. Developmental cell biology is rapidly emerging as the branch of the subject producing some of the most exciting revelations about the way the complex pattern of differentiated tissues becomes organized.
Communication
The developing multicellular organism becomes divided into groups of cells cooperating as tissues. These must selectively adhere to one another to remain as organs, and their component cells must also be able to communicate with each other if division of labour is to occur effectively. This they do by a number of devices, of which one of the most important is the nervous system, where signals are sent by chemical neurotransmitters from one neuron to other cells. Hormones, protein or steroid, also have profound chemical effects on developmental processes, including the acquisition of secondary sexual characteristics, by activating different genes in the DNA. Circulating hormones may also trigger secondary signalling pathways. Local hormones play important roles in adjacent cellular activity, as may other chemicals that operate by chemotaxis or via chemical attractants. Signalling via cell surface molecules also occurs in the immune system, which is based on cellular signals and responses that activate special B and T cells in the lymphoid system, using either humoral or cellmediated mechanisms.
This is important in enabling the organism to survive infection. Growth factors and cellular adhesion molecules may also affect the patterns of differentiation of cells in the formation, and then the maintenance, of organs. This may involve the assembly of the cell–cell junctions, described earlier, which keep the adjacent cells associated together in particular arrangements.
The interplay of chemical signals between the cells of the same or different organs produces an orchestration of cellular activity resulting in a successful, functional organism. The healthy organism, therefore, is dependent on the effective functioning of the cells that make up its organs.
Further Reading
- Alberts B, Bray D, Johnson Aet al. (1998) Essential Cell Biology: An Introduction to the Molecular Biology of the Cell. New York: Garland.
- Darnell J, Lodish H and Baltimore D (1990)
- Lewin B (1983) Genes. New York: Wiley. Rensberger B (1996).
Published date : 10 May 2014 02:49PM